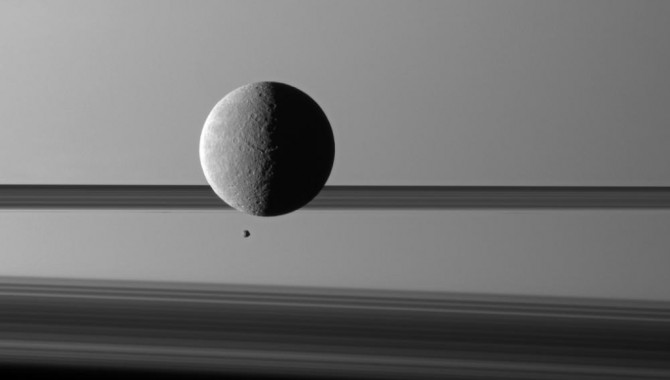
By Brent Buffington
Cassini-Huygens, a joint mission between NASA, the European Space Agency, and the Italian Space Agency, has roamed the Saturnian system for the better part of six and a half years.
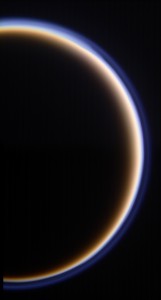
Titan’s golden, smog-like atmosphere and complex layered hazes appear to Cassini as a luminous ring around the planet-sized moon.
Photo Credit: NASA/JPL
Data from the Cassini spacecraft have led to discoveries that include water ice and vapor geysers in the south polar region of the small moon Enceladus; an active hydrocarbon hydrological cycle (including liquid methane/ethane lakes, dendritic channels, dunes, clouds, and possible precipitation) on Titan; verification of the continued existence of a perplexing hexagon-shaped structure near Saturn’s north pole, first observed by Voyager 1; and a number of new, highly complex, and dynamic structures in our solar system’s most massive and diverse ring system. These and many other discoveries have been made possible by highly successful spacecraft operations and subsystem maintenance, sequencing, instrument monitoring, risk management, and navigation of the most complex gravity-assist tour in the history of unmanned spaceflight.
A Rich Scientific Harvest
Cassini’s four-year prime mission, from July 2004 to July 2008, sustained a staggering pace of scientific discovery, generating more than one thousand independent publications in prominent science and engineering journals. During the prime mission—which included 45 encounters with Titan, 4 with Enceladus, and 6 with other icy satellite flybys; 75 Saturn orbits; 161 planned maneuvers (112 executed); and the many science sequence and flight software updates—Cassini averaged two key events per week. Through all these activities, spacecraft subsystems and instruments remained healthy, substantial consumables margins were maintained, and power levels from the radioisotope thermoelectric generators allowed for many more years of science operations.
The continuing quality of scientific return and the spacecraft’s excellent state of health led NASA Headquarters to grant it two mission extensions. The first, the Equinox mission, was a 2.25-year extension from July 2008 to October 2010 that carried Cassini through Saturn’s northern-hemispheric vernal equinox in August 2009. Even though this mission was technically an extension, it was similar in scope and funding to the prime mission, with science observations and the related navigation and spacecraft operations continuing at the same or greater pace. In all, the Equinox mission included 28 Titan flybys, 8 Enceladus and 3 other icy satellite flybys, 64 Saturn orbits, and 104 planned maneuvers (70 executed).
The second extension, the Solstice mission, required a radical change in design methodology. Its overriding scientific goal is to reach Saturn’s northern summer solstice in May 2017, more than doubling the total mission duration, with only 20 percent of the propellant available at the beginning of the prime mission. Furthermore, an expected 40 percent reduction in staffing would limit the frequency of key events as well as the complexity of the observational sequences.
Designing the Solstice Trajectory
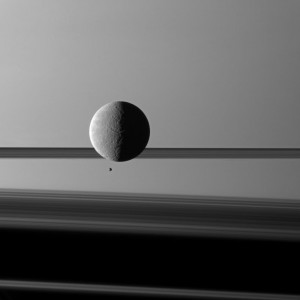
Saturn’s moon Rhea looms “over” a smaller and more distant Epimetheus.
Photo Credit: NASA/JPL/Space Science Institute
The process of designing a trajectory is bound by the laws of physics and driven by scientific intrigue. The goal is to maximize the number of high-quality scientific observations and measurements while minimizing propellant expenditure and adhering to operational, spacecraft, and environmental constraints. Scientists from five discipline working groups (Saturn, rings, Titan, icy satellites, and magnetosphere and plasma) developed more than forty science objectives to guide the Solstice mission design and addressed two major themes: seasonal/temporal change and new questions that have arisen since the start of the mission.
The enabling mechanism for the complicated mission design of the Cassini trajectories is a concept understood for over a century and employed in a number of missions during the past forty years: the gravity assist. A gravity assist entails a spacecraft using a massive, intermediate, moving celestial body to significantly modify its trajectory. Depending on the flyby speed and distance, and how the spacecraft flies by the large gravitating body (above/below, behind/in front), the spacecraft’s orbit size, period, energy, inclination, and distance relative to the central body can be altered in an incremental and predictable manner such that a wide range of geometries can be attained to meet myriad, often disparate, scientific goals.
Given the high velocities with which Cassini encounters the various moons of Saturn, Titan is the only Saturnian satellite massive enough to significantly alter the spacecraft’s trajectory. To quantify the significance of Titan, consider this: the amount of propellant onboard a spacecraft is often expressed in terms of how much the propellant can change the velocity of the spacecraft in meters per second (m/s), or “delta v” (Δv). After entering into orbit around Saturn in July 2004 (which required a 627 m/s Saturn orbit insertion maneuver and a 393 m/s maneuver to raise periapsis, the lowest point of the orbit), Cassini possessed a total Δv capability of approximately 754 m/s. By contrast, a single Titan flyby can provide a change in the spacecraft’s velocity in excess of 800 m/s. In the course of more than seventy Titan flybys to date, the large moon has imparted to Cassini a total Δv of more than 50,000 m/s.
The core of designing trajectories resides in orbital mechanics—the fundamental understanding of how objects interact with one another in outer space—and the ability to model these interactions accurately and quickly enough to evaluate the efficiency and scientific quality of many different routes.
For any given Titan flyby, several options exist for the post-flyby spacecraft orbit(s) depending on how we choose to fly by Titan. These choices increase geometrically as the number of Titan flybys increases, quickly overwhelming any attempts to design an optimal trajectory computationally. The immense number of possible tours creates both opportunities and difficulties.
To prune the multitude of options associated with a seven-year trajectory comprising more than fifty Titan flybys, John Smith and I (the tour designers) conducted parametric studies to determine how various science objectives fit with one another: which were complementary, mutually exclusive, and cheap or expensive both in propellant and time, and which made more sense to carry out at specific times in the tour. Guided by these studies, we built a handful of end-to-end tours, attempting to incorporate as many requested observations as possible for more than two hundred scientists (from the United States and seventeen European nations) to assess. The optimization of tours hinged on the communication between the tour designers and scientists, with the tour quality and complexity evolving as the interaction of scientific objectives became better understood and the geometric constraints defining them became refined. Based on the scientists’ evaluations—what characteristics of each tour were good, bad, and ugly—we would adapt our strategies and develop a fresh crop of candidate trajectories to be released and evaluated three to four months later. Survival of the best trajectory traits and the development of new traits was Darwinism at its finest.
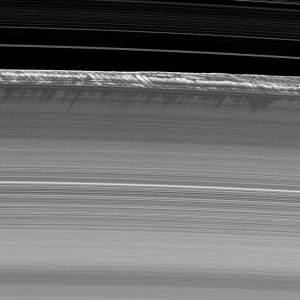
Vertical structures, among the tallest seen in Saturn’s main rings, rise abruptly from the edge of Saturn’s B ring to cast long shadows on the ring.
Photo Credit: NASA/JPL/Space Science Institute
The core of designing trajectories resides in orbital mechanics—the fundamental understanding of how objects interact with one another in outer space—and the ability to model these interactions accurately and quickly enough to evaluate the efficiency and scientific quality of many different routes. But on many levels, trajectory design is as much an art as a science. We started with a set of initial conditions (the ending conditions of the Equinox mission) and a blank canvas of space and time in front of us. Much like a painter, we have a finite number of “colors” in our palette available to paint our masterpiece—in our case, resonant, non-resonant, and pi-transfers; equatorial and inclined orbits; and inbound and outbound Titan flybys. The way in which we combined these colors was motivated by high-priority scientific objectives, guided by experience-based intuition and flashes of inspiration, and governed by spacecraft capabilities (control authority, available propellant), environmental constraints (Saturn’s rings, Titan’s atmosphere, Enceladus’ plume, solar conjunction), operational intensity constraints (time-of-flight between flybys, maneuver frequency), ground-system limitations, and a finite mission duration. Within those constraints, we had creative freedom, each brush stroke (that is, each Titan flyby) giving rise to certain geometric opportunities while precluding others, our choices an attempt to create the most aesthetically (scientifically) pleasing painting possible.
Achieving consensus among more than two hundred scientists from five different disciplines’ working groups vying for observation time needed to carry out the scientific investigations to which they have dedicated their lives was not always a simple matter. With twelve instruments on Cassini, there were also numerous and competing interests within the discipline working groups. Some objectives required the spacecraft’s orbit plane to be the same as Saturn’s ring plane (which is also very close to the orbit plane of the larger inner satellites); other objectives required medium or highly inclined orbits. Additional examples of objective-driven geometry included being close to or far from Saturn, placing ground tracks over specific features or regions of satellites, flying during a solar phase, and orientating the line of apsides1 with respect to the Saturn–Earth line. To further complicate matters, a single objective could require many different types of observational geometries, sometimes from multiple instruments, as well as repeated observations to determine temporal variation.
After two years of development and numerous iterations between the tour designers and the scientists (the number of iterations was limited by schedule deadlines), the project selected a single tour that best addressed the majority of the high-priority science objectives and fit within established operational and safety constraints. We then spent a few additional months implementing a number of small tweaks to further optimize science return. A final reference trajectory, the end product of the tour-design process, was delivered to the project in July 2009.
The Solstice mission, from October 2010 to September 2017, includes 54 Titan flybys, 11 Enceladus and 8 other icy satellite flybys, 155 Saturn orbits, and 206 planned maneuvers. It will extend Cassini’s operational lifetime past Saturn’s northern summer solstice to increase the observed temporal baseline beyond two Saturnian seasons. In addition, to satisfy the NASA Planetary Protection Office’s requirement to minimize the probability of biological contamination of the Saturnian moons, Enceladus and Titan, from inadvertent spacecraft impact before control of the spacecraft is lost (that is, when the gas tanks are empty), a Saturn-impact, end-of-mission scenario2 was incorporated into the Solstice mission. Before the spacecraft’s fate is sealed, however, the mission will implement one last encore, aptly referred to as the “proximal orbits.”
Prior to impact, a spectacular series of twenty-two orbits will be executed; during each one, Cassini will pass through a 1,850-mile gap (believed to be clear of any debris harmful to the spacecraft) interior to Saturn’s innermost ring, just above the cloud tops of Saturn, at speeds in excess of 76,000 mph. Finally, on the twenty-third orbit, one last high-altitude Titan flyby will alter the spacecraft’s trajectory such that Cassini will impact Saturn four days later, trading its temporary roaming residency in the Saturnian system for one of a more permanent stature via a fiery collision into Saturn. The elegance of this spacecraft-disposal option is that Saturn impact is guaranteed regardless of whether the spacecraft survives the first (or any) proximal orbit-ring plane crossing—no further propulsive maneuvers are necessary. And, en route, unique science opportunities beyond the initial scope of the Cassini project will be available. A spectacular ending to a spectacular mission.
Note: This work was carried out at the Jet Propulsion Laboratory, California Institute of Technology, under a contract with the National Aeronautics and Space Administration. © California Institute of Technology. Government sponsorship acknowledged.
About the Author
![]() |
Brent Buffington is a member of the Outer Planets Mission Analysis Group at the Jet Propulsion Laboratory and was part of the trajectory design teams responsible for the Cassini Equinox and Solstice missions. Currently, he is an orbit determination analyst on the Cassini navigation team. |
1The line connecting the points of the orbit furthest from and closest to the planet.
2This spacecraft-disposal option has been reviewed but not formally approved by NASA Headquarters